
The Ashbaugh group uses statistical mechanics and molecular simulations to uncover the thermodynamic forces that drive molecular self-assembly, from surfactants to bio-inspired supramolecules, and the structures formed. Our goal is to provide molecular-scale insights to guide experimentalists to design new functional nano-materials from the bottom-up. Group research focuses on two levels of analysis: nano-scale simulations of assembled soft-materials, like micelles and cavitand complexes, to examine their structure and synergistic interactions between constituents; and simulations of simplified systems to directly probe thermodynamic forces, like hydrophobic interactions, isolated from competing interactions that complicate interpretation of larger-scale assembly. Our work has benefited from collaborations with experimental colleagues working to develop new materials with applications from drug delivery, to gas separations, to oil spill remediation.
Micelle Assembly. Surfactants are schizophrenic molecules typically composed of a water-soluble polar (hydrophilic) head group and water-insoluble non-polar (hydrophobic) tail group. The aggregation of surfactants into micelles is a remarkable demonstration of the utility of the hydrophobic effect at driving non-specific self-assembly phenomena in aqueous solution. The ability to tune the concentration range over which surfactant micellization occurs, their aggregation number, and the shape of the structures formed ultimately determines the applications they may be used, from solubilizing agents (as in soaps) to templates for formation of inorganic materials like zeolites. Recent publicity on the use of surfactants has focused on their application as dispersants for breaking down oil spill slicks into small droplets that can be remediated by natural degradation processes. Specific concerns have been raised about the toxicity of dispersants in the environment, while similar concerns could be leveed against surfactants employed in fracking fluids. A key towards designing oil field surfactant formulations is dissecting the roles of the head and tail groups in stabilizing micelles subjected to the extremes of temperature and pressure faced in environments ranging from the ocean surface, to the sea floor, to reservoir rock formations. The Ashbaugh group is subsequently using molecular simulations to examine the thermodynamics of surfactant assembly under extreme conditions to address these challenges
While surfactant aggregation and protein folding both
rely on hydrophobic interactions of drawing together the
individual surfactant chains and collapsing the
polypeptide into a globular state, these assemblies
exhibit distinct responses to increasing pressure. Proteins
tend to denture with increasing pressure. Micelles, on
the other hand, initially fall apart with increasing pressure
and subsequently reform with further increases in
pressure, as indicated by a maximum in the surfactant’s
critical micelle concentration (CMC) at pressure of
~1000 atm. This pressure re-entrant micellization
behavior can resultantly impact surfactant efficacies
under extreme conditions. From a thermodynamic point of
view, a maximum in the CMC is indicative of a crossover of
the surfactant volumes of micellization from positive to
negative values with increasing pressure. Using
molecular simulations the Ashbaugh group has examined volumes of micellization (determined as the partial molar volume difference between micellized and monomeric surfactants) for a range of surfactants with varying head group chemistries, from nonionic, to anionic, and cationic, as a function of pressure. Our simulations directly observe the change in sign from positive to negative volumes for all the surfactants examined in good agreement with experiment, with the volume crossing zero in the neighborhood of ~1000 atm where the maximal CMC is expected. We were able to break the simulated volumes of micellization into head and tail group contributions using Kirkwood-Buff theory, demonstrating for the charged head groups that the hydrophobic tail largely determines the crossover point. For the nonionic the head group also surfactants on the other hand, plays a significant role in determining the pressure at which the maximal CMC is observed, moderated by dehydration of the micelle corona with increasing pressure.
Supramolecular Assembly. Like surfactants, cavitands can assemble in water to form nano-structured materials, however, the rules guiding their assembly can be distinct. Deep-cavity octaacid (OA) cavitands, for example, are bowl-shaped molecules with water-soluble groups (like carboxylic acids) coating the exterior of the non-polar bowl, which has a depth and diameter of ~8Å. While cavitands do not aggregate on their own, they can serve as hosts to hydrophobic guests that can fit within the non-polar bowl. The host-guest supramolecular complexes formed can subsequently adopt a range of different host-guest stoichiometries and structures that depend on the synergistic interactions between the co-assembling species. For example, n-alkanes shorter than nonane form one-to-two (1:2) or one-to-one host-guest complexes with OA, while longer n-alkanes for 2:1 dimeric capsules that sequester the guests completely from water. Working with an experimental collaborator, Prof. Bruce Gibb (Chemistry at Tulane) who specializes in cavitand synthesis and characterization, we have been simulating host-guest complexation and the structures assumed by the host and guest within the complex. The potential applications for these systems include: drug delivery vehicles, hydrocarbon gas separations, yocto-liter scale reactors, synthetic receptors, and gelling agents. The ability to tune host and guest interactions, by changing host/guest chemistries or varying the solution conditions, is essential to designing these supramolecular assemblies for specified applications.easing pressure.
Ongoing research on cavitand host-guest complexes in the Ashbaugh group has split in two directions: examining the packing and conformation of n-alkanes confined within OA 2:1 complexes; and examining the interplay between n-alkane chain length and the host-guest aggregation state of complexes with the related host tetra-endo-methyl octaacid (TEMOA). Our simulations of n-alkanes in OA dimers show a progression of
guest conformational motifs with increasing chain length, from
extended, to helical, to hairpin-like, to spinning top-like.
Subsequent predictions of changes in the encapsulated guest
1H-NMR chemical shifts determined by Gauge Invariant Atomic
Orbital calculations are strongly correlated with experimental
measurements, providing evidence that the simulations are
representative of the actual guest conformations. Our simulations
also provide experimentally unavailable detail on the host-guest
interactions and free energies that dictate the stable
conformations to guide optimization of guest packing within the
yocto-liter sized complex volumes. Separate simulations of
n-alkanes threaded through carbon nanotubes have demonstrated
the role of the width of the confining pore on the equilibrium between the extended and helical conformers.
Solvation Thermodynamics. While the individual surfactant, polymer, biomolecule, and supramolecular chemical details are essential to understanding assembly, the solvent within which these processes take place also plays a critical role in guiding assembly. The solvent stage upon which all the assembly processes described above is water, with hydrophobic effects driving non-specific association of the non-polar units and the hydration of polar groups maintaining solubility of the assembled complexes. A third research theme within the Ashbaugh group is therefore focused on understanding solvation phenomena, with the aim of understanding solvent thermodynamic contributions to assembly.
While hydrophobic interactions are frequently invoked as the primary thermodynamic driving force for assembly in aqueous solution, direct experimental probes of the water-mediated interaction between nonpolar species is lacking. While these interactions in principal can be determined from scattering experiments, the low solubility of non-polar species makes measurement of the scattering intensity untenable. Alternately, osmotic pressure measurements of gases at low concentrations in water can provide information on water-
mediated interactions. Evaluation of second osmotic virial
coefficients from simulations, however, has been challenging as
a result of the long simulation times required and difficulties in
evaluation of the long-ranged integrals over the solute radial
distribution functions (RDFs) involved. Utilizing new approaches
we have attacked this problem on two fronts. In collaboration with
Prof. Lawrence Pratt we demonstrated that the second osmotic
coefficients of argon-sized hard spheres in water could be
directly evaluated from their RDFs in water from long simulations
of sufficiently large systems. These calculations successfully
demonstrated that the interaction between argon-sized species is
endothermic (that is interactions get stronger), indicative of
entropically driven association between nonpolar gases in water.
Extensive follow up simulations carried out in the Ashbaugh group on the interactions between methanes in water demonstrated that methane second osmotic virial coefficients exhibit a hyperbolic saddle in the temperature-pressure plane, analogous to the behavior of the surfactant CMCs. More importantly, we validated our osmotic virial coefficients evaluated from RDFs against an independent formulation based on the concentration dependence of the chemical potential, confirming for the first time that the new theoretical approaches to evaluating osmotic coefficients yield accurate values for the models employed. Our simulations, however, still presage reliable experimental osmotic virial coefficient determination. Nevertheless, we have recently extended methods developed in our methane simulations in to describe the differences between the experimental free energies of ethane and methane in water, which Ben-Naim argued can provide insights into hydrophobic interactions. Our simulations have illustrated the strengths and shortcomings of Ben-Naim’s approach and support the accuracy of simulation predictions of molecular-level hydrophobic interactions.
While the studies described above have provided new models and approaches to describing molecular-scale hydration and interactions, the bottom-up construction of new materials via self-assembly necessarily encompasses interactions over multiple length scales, from the molecular to the meso-scopic level. Molecular simulations become computationally prohibitive with increasing assembly sizes. Statistical thermodynamic theories of how interactions vary with changes in length scale, however, can bridge the molecular to meso-
scopic scales. Hydrophobic interactions, for example are
frequently ascribed to depend on specific water structures on the
molecular scale, and on the surface tension for meso-scopic
and larger surfaces. Confirmation of the predicted scaling
behavior between length scales is largely untested, however, due
to the large-scale simulation difficulties described above. As a
step towards validating surface tension based theories, we
focused on testing the scaling behavior rather than its application
to interactions in liquid water. To this end, we simulated the
interactions between hard plates in a two-dimensional Lennard-
Jones fluid. This simplification allowed us overcome many of
the computational difficulties of examining interactions in water
while retaining a solvent with molecular degrees of freedom.
These simulations demonstrated that when solute surfaces are
within a critical separation the liquid between them becomes unstable and spontaneous evaporates. This evaporation event coincides with the onset of strong interactions between the surfaces driven by the surface tension attempting to reduce the contact between the bubble confined between plates and the bulk solvent. We were able to examine a wide range of state points and plate sizes that confirmed the predictions of the scaling theory, with minor corrections to account for the molecular nature of the solvent. More importantly our simulations were able for the first time to probe values of the dimensionless group that controls these solvent-mediated interactions, the cavitation number, relevant to experiment.
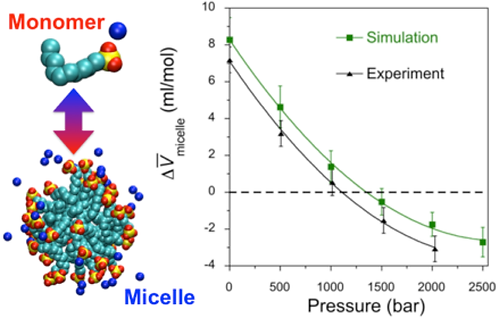



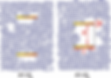